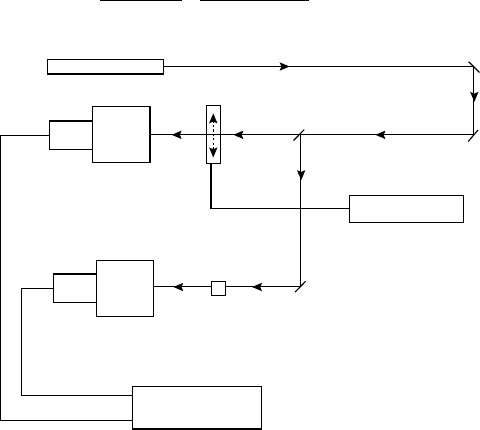
and the local fields F
qv
p
take into account the difference
between the actual field seen by the molecules and the
applied field E
qv
p
. There are several experimental techniques
that can be employed to evaluate NLO coefficients
b, g, w
(2)
, and w
(3)
of polymeric materials.
At present, polymers that exhibit large nonresonant op-
tical nonlinearities are of considerable interest in scientific
and industrial circles. Organic polymers offer significant
tailoring flexibility in that their chemical structures can be
modified to optimize the microscopic NLO response at the
molecular level. Furthermore, at the bulk and microstructure
level polymers can be processed as fibers, thin films in
oriented or unoriented forms, glasses, and gels. NLO pro-
cesses provide important functions of frequency conversion
(for example, frequency doubling to increase the density of
data storage), light controlled by electric field and even
optical processes such as light controlled by light, the mani-
festation of which can be utilized to build photonic devices.
A photonic device utilizes photons instead of electrons to
acquire, process, transmit, and store information [3]. In
order to be successfully applied in light-wave technology
and optical circuitry a material should satisfy many criteria:
easy processing, high transparency, high physical, mechan-
ical, thermal, electrical, and chemical stability, compatibil-
ity with the other materials used for microelectronics, high
optical power damage threshold, high optical nonlinearity,
and reasonably low cost. Polymeric materials may be, per-
haps, the first to combine most of these properties, avoiding
stringent tradeoffs.
Concepts of optical computing and optical signal and
image processing have been developed utilizing NLO pro-
cesses to perform the functions of frequency conversions,
light modulation, optical switching, optical logic, optical
memory storage, and optical limiter functions. Devices
performing these functions utilize two important mani-
festations: frequency conversion and refractive-index
modulation. In the case of the second-order NLO effects
refractive-index modulation is produced by application of
an external electric field. Using second-order effects poly-
meric materials may find applications such as in second-
harmonic generation, high-density data storage, and electro-
optic spatial light modulation in the near future. As far as the
third-order NLO effects are concerned, the refractive index
is modulated by controlling the intensity of the optical field,
and it provides the mechanism for optical switching and
optical bistability. The main advantage of using all-optical
processing is the gain of speed and gain in connectivity.
Polymeric materials posses fast NLO response and also can
be fabricated in the form of fibers and channel waveguides
to satisfy the necessary requirements. In addition, an im-
portant third-order NLO phenomenon is two-photon absorp-
tion (TPA) in which a molecule can simultaneously absorb
two photons, when irradiated by intense laser pulses. Since
the availability of femtosecond (fs) laser in the 1990s, a
great deal of work has been done for developing the efficient
two-photon absorbing materials. Such TPA materials can be
employed for various photonic applications including 3-D
optical data storage, 3-D microfabrication, photodynamic
therapy, and optical power limiting. In this chapter, we
discuss the topics related to certain theoretical aspects,
concepts of material design, and evaluation techniques
for second- and third-order NLO polymers as well as for
two-photon absorbing organic and polymeric materials.
49.2 MEASUREMENTS OF b OF POLYMERS
Organic polymers, being amorphous, do not exhibit any
second-order NLO effect, even though the molecules them-
selves are acentric. Therefore, in order to observe any sec-
ond-order NLO effect, the isotropy of the medium has to be
perturbed. This is usually accomplished by application of a
strong dc electric filed. In the liquid phase, measurements
are made by the technique known as electric-field-induced
second-harmonic (EFISH) generation [5]. In this technique
the solution is contained in a wedge-shape fused silica cell.
The cell is sandwiched between two electrodes. A large dc
voltage pulse is applied to the electrodes to disturb the
average molecular orientation. At the same time the probing
laser beam also is incident on the cell. The emerging sec-
ond-harmonic generation (SHG) signal at 2v from the cell is
recorded, as the cell is translated across the laser beam (Fig.
49.1). The NLO polarization responsible for the EFISH
process can be expressed as [3]
P
i
(2v) ¼ w
(3)
ijkl
( 2v ;v,v,0)E
v
j
E
v
k
E
0
l
: (49:4)
As the cell is moved across the incident laser beam, the optical
path inside the cell varies linearly with the translated distance,
so the resulting SHG signal exhibits an oscillatory behavior.
The intensity of the EFISH signal can be approximated by
I
2v
¼
8p(2v)
2
n
2
v
n
2
2v
c
2
«
2
0
sin
2
[Dk(l=2)]
[Dk(l=2)]
2
jw
(3)
j
2
l
2
I
2
v
, (49:5)
Laser
Monochromator
Monochromator
Reference
BOXCAR
Beam Splitter
EFISH Cell
PMT
PMT
Pulsed HV
Power Supply
FIGURE 49.1. Schematics of experimental arrangement of
electric-field-induced second-harmonic generation.
796 / CHAPTER 49