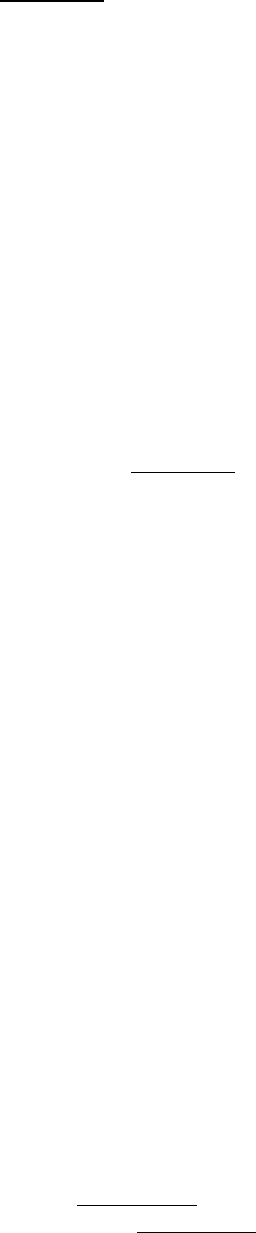
both strands. The shortened piece of DNA is then ligated to form a circle that is missing a short length of DNA about the
restriction site.
Substitutions: Oligonucleotide-Directed Mutagenesis.
Mutant proteins with single amino acid substitutions can be readily produced by oligonucleotide-directed mutagenesis
(Figure 6.36). Suppose that we want to replace a particular serine residue with cysteine. This mutation can be made if (1)
we have a plasmid containing the gene or cDNA for the protein and (2) we know the base sequence around the site to be
altered. If the serine of interest is encoded by TCT, we need to change the C to a G to get cysteine, which is encoded by
TGT. This type of mutation is called a point mutation because only one base is altered. The key to this mutation is to
prepare an oligonucleotide primer that is complementary to this region of the gene except that it contains TGT instead of
TCT. The two strands of the plasmid are separated, and the primer is then annealed to the complementary strand. The
mismatch of 1 base pair of 15 is tolerable if the annealing is carried out at an appropriate temperature. After annealing to
the complementary strand, the primer is elongated by DNA polymerase, and the double-stranded circle is closed by
adding DNA ligase. Subsequent replication of this duplex yields two kinds of progeny plasmid, half with the original
TCT sequence and half with the mutant TGT sequence. Expression of the plasmid containing the new TGT sequence
will produce a protein with the desired substitution of serine for cysteine at a unique site. We will encounter many
examples of the use of oligonucleotide-directed mutagenesis to precisely alter regulatory regions of genes and to produce
proteins with tailor-made features.
Insertions: Cassette Mutagenesis.
In another valuable approach, cassette mutagenesis, plasmid DNA is cut with a pair of restriction enzymes to remove a
short segment (Figure 6.37). A synthetic double-stranded oligonucleotide (the cassette) with cohesive ends that are
complementary to the ends of the cut plasmid is then added and ligated. Each plasmid now contains the desired mutation.
It is convenient to introduce into the plasmid unique restriction sites spaced about 40 nucleotides apart so that mutations
can be readily made anywhere in the sequence.
Designer Genes.
Novel proteins can also be created by splicing together gene segments that encode domains that are not associated in
nature. For example, a gene for an antibody can be joined to a gene for a toxin to produce a chimeric protein that kills
cells that are recognized by the antibody. These immunotoxins are being evaluated as anticancer agents. Entirely new
genes can be synthesized de novo by the solid-phase method. Furthermore, noninfectious coat proteins of viruses can be
produced in large amounts by recombinant DNA methods. They can serve as synthetic vaccines that are safer than
conventional vaccines prepared by inactivating pathogenic viruses. A subunit of the hepatitis B virus produced in yeast is
proving to be an effective vaccine against this debilitating viral disease.
6.4.2. Recombinant DNA Technology Has Opened New Vistas
Recombinant DNA technology has revolutionized the analysis of the molecular basis of life. Complex chromosomes are
being rapidly mapped and dissected into units that can be manipulated and deciphered. The amplification of genes by
cloning has provided abundant quantities of DNA for sequencing. Genes are now open books that can be read. New
insights are emerging, as exemplified by the discovery of introns in eukaryotic genes. Central questions of biology, such
as the molecular basis of development, are now being fruitfully explored. DNA and RNA sequences provide a wealth of
information about evolution. Biochemists now move back and forth between gene and protein and feel at home in both
areas of inquiry.
Analyses of genes and cDNA can reveal the existence of previously unknown proteins, which can be isolated and
purified (Figure 6.38A). Conversely, purification of a protein can be the starting point for the isolation and cloning of its
gene or cDNA (Figure 6.38B). Very small amounts of protein or nucleic acid suffice because of the sensitivity of
recently developed microchemical techniques and the amplification afforded by gene cloning and the polymerase chain