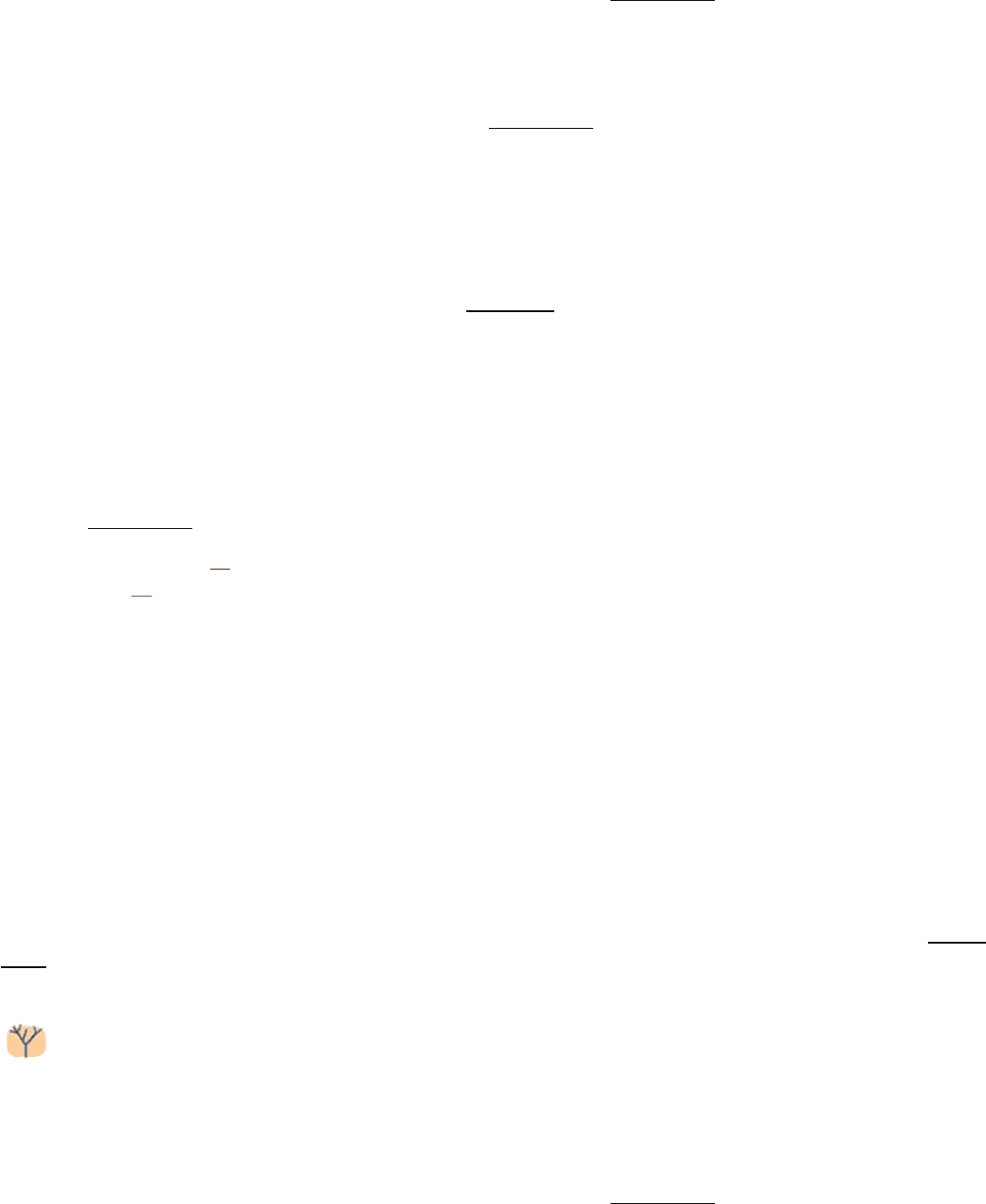
pore are filled with water, and a K
+
ion can fit in the pore without losing its shell of bound water molecules.
Approximately two-thirds of the way through the membrane, the pore becomes more constricted (3-Å diameter). At that
point, any K
+
ions must give up their water molecules and interact directly with groups from the protein. The channel
structure effectively reduces the thickness of the membrane from 34 Å to 12 Å by allowing the solvated ions to penetrate
into the membrane before the ions must directly interact with the channel (Figure 13.23).
For potassium ions to relinquish their water molecules, other polar interactions must replace those with water. The
restricted part of the pore is built from residues between the two transmembrane helices (which correspond to segments
S5 and S6 in the sodium channel). In particular, a five-amino-acid stretch within this region functions as the selectivity
filter that determines the preference for K
+
over other ions (Figure 13.24). The stretch has the sequence Thr-Val-Gly-Tyr-
Gly, which is nearly completely conserved in all K
+
channels and had already been identified as a signature sequence
useful for identifying potential K
+
channels. This region lies in a relatively extended conformation and is oriented such
that the peptide carbonyl groups are directed into the channel, facilitating interaction with the potassium ions.
Potassium channels are 100-fold as permeable to K
+
as to Na
+
. How is this high degree of selectivity achieved? The
narrow diameter (3 Å) of the selectivity filter of the potassium channel enables the filter to reject ions having a radius
larger than 1.5 Å. However, a bare Na
+
is small enough (Table 13.2) to pass through the pore. Indeed, the ionic radius of
Na
+
is substantially smaller than that of K
+
. How then is Na
+
rejected?
We need to consider the free-energy cost of dehydrating the Na
+
and K
+
ions, given that they cannot pass through this
part of the channel bearing a retinue of water molecules. The key point is that the free-energy costs of dehydrating these
ions are considerable [Na
+
, 72 kcal mol
-1
(301 kJ mol
-1
), and K
+
, 55 kcal mol
-1
(203 kJ mol
-1
)]. The channel pays the
cost of dehydrating K
+
by providing compensating interactions with the carbonyl oxygen atoms lining the selectivity
filter. However, these oxygen atoms are positioned such that they do not interact very favorably with Na
+
, because it is
too small (Figure 13.25). The higher cost of dehydrating Na
+
would be unrecovered, and so Na
+
would be rejected. The
ionic radii of oxygen, potassium, and sodium are 1.4, 1.33, and 0.95 Å, respectively. Hence a ring of oxygen atoms
positioned so that the K
+
O distance is 2.73 Å (1.4 + 1.33 Å) would be optimal for interaction with K
+
compared with
the shorter Na
+
O bonds (0.95 + 1.4 = 2.35 Å) optimal for interaction with Na
+
. Thus, the potassium channel avoids
closely embracing Na
+
ions, which must stay hydrated and hence are impermeant.
13.5.7. The Structure of the Potassium Channel Explains Its Rapid Rates of Transport
In addition to selectivity, ion channels display rapid rates of ion transport. A structural analysis provides an appealing
explanation for this proficiency. The results of such studies revealed the presence of two potassium-binding sites in the
constricted regions of the potassium channel that are crucial for rapid ion flow. Consider the process of ion conductance.
One K
+
ion proceeds into the channel and through the relatively unrestricted part of the channel. It then gives up most or
all of its coordinated water molecules and binds to the first site in the selectivity filter region, a favorable binding site. It
can then jump to the second site, which appears to have comparable binding energy. However, the binding energy of the
second site presents a free-energy barrier, or trap, preventing the ion from completing its journey; there is no energetic
reason to leave the second ion-binding site. However, if a second ion moves through the channel into the first site, the
electrostatic repulsion between the two ions will destabilize the initially bound ion and help push it into solution (Figure
13.26). This mechanism provides a solution to the apparent paradox of high ion selectivity (requiring tight binding sites)
and rapid flow.
The structure determined for K
+
channels is a good start for considering the amino acid sequence similarities, as
well as the structural and functional relations, for Na
+
and Ca
2+
channels because of their homology to K
+
channels. Sequence comparisons and the results of mutagenesis experiments have also implicated the region between
segments S5 and S6 in ion selectivity in the Ca
2+
channels. In Ca
2+
channels, one glutamate residue of this region in
each of the four units plays a major role in determining ion selectivity. The Na
+
channel's selection of Na
+
over K
+
depends on ionic radius; the diameter of the pore is sufficiently restricted that small ions such as Na
+
and Li
+
can pass
through the channel, but larger ions such as K
+
are significantly hindered (Figure 13.27).