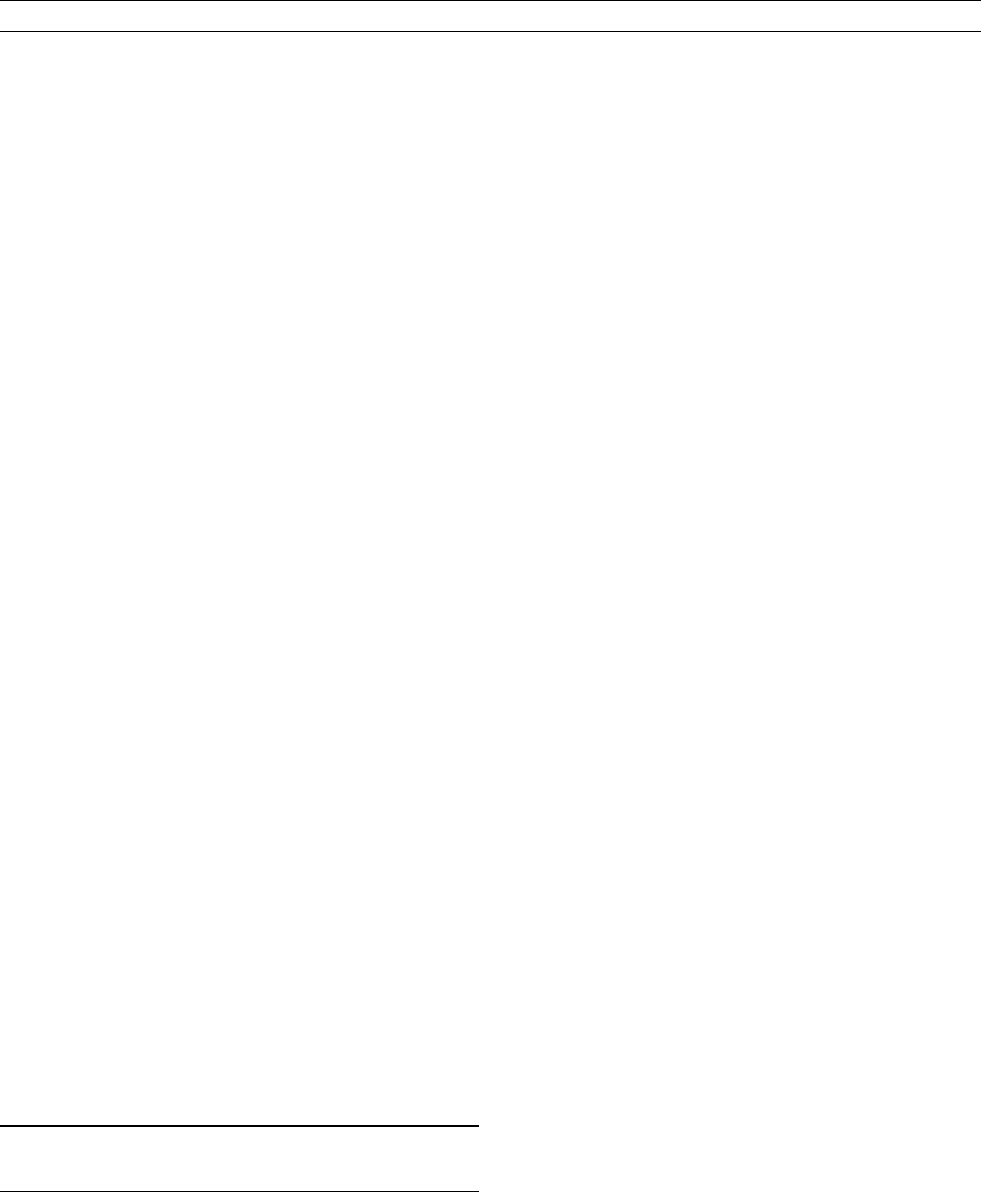
although this is still a matter of debate, oceanic basalts could provide a
record of the magnetic field intensity variations within polarity intervals,
as suggested by the worldwide occurrence of small scale magnetic var-
iations (called “tiny wiggles”) within the main magnetic anomaly pat-
tern (Cande and Kent, 1992). In any case, it should be mentioned
again that the local crustal architecture is probably complex (Karson
et al., 2002) and is a major factor to take into account in the analysis
of marine magnetic signals.
Julie Carlut and Helene Horen
Bibliography
Cannat, M.C. et al., 1995. Thin crust, ultramafic exposures, and rugged
faulting patterns at the Mid-Atlantic Ridge (22
N–24
N). Geology,
23:149–152.
Cande, S.C., and Kent, D.V., 1992. Ultrahigh resolution marine mag-
netic anomaly profiles—a record of continuous paleointensity var-
iations. Journal of Geophysical Research, 97(B11): 15075–15083.
Dick, H.J.B. et al., 2000. A long in situ section of the lower ocean
crust: results of ODP Leg 176 drilling at the Southwest Indian
Ridge. Earth and Planetary Science Letters, 179:31–51.
Dunlop, D., and Ozdemir, O., 1997. Rock Magnetism. Cambridge:
Cambridge University Press, 573 pp.
Kent, D.V. et al., 1978. Magnetic properties of dredged oceanic gab-
bros and the source of marine magnetic anomalies. Geophysical
Journal of the Royal Astronomical Society, 55: 513–537.
Karson, J.A. et al., 2002. Structure of the uppermost fast spread ocea-
nic crust exposed at the Hess Deep Rift: implications for subaxial
processes at the East Pacific Rise. Geochemistry, Geophysics,
Geosystems, 3: DOI:10.1029/2001GC000155.
Klitgord, K.D. et al., 1975. An analysis of near bottom magnetic
anomalies: seafloor spreading and the magnetized layer. Geophysi-
cal Journal of the Royal Astronomical Society, 43: 387–424.
Morley, L., and La Rochelle, A., 1963. Paleomagnetism and the dating
of geological events. Transactions of the Royal Society of Canada,
1: App 31.
O’Reilly, W., 1984. Rock and Mineral Magnetism. London: Blackie,
220 pp.
Oufi, O. et al., 2002. Magnetic properties of variably serpentinized
abyssal peridotites. Journal of Geophysical Research, 107(B5):
10.1029/2001JB000549.
Smith, G.M., and Banerjee, S.K., 1986. Magnetic structure of the
upper kilometer of the marine crust at deep sea drilling project hole
504B, Eastern Pacific Ocean. Journal of Geophysical Research,
91(B10): 10337–10354.
Vine, F.J., and Matthews, D.H., 1963. Magnetic anomalies over ocea-
nic ridges. Nature, 199: 947
–949.
Zhou, W. et al., 2000. Variable Ti-content and grain size of titanomag-
netite as a function of cooling rate in very young MORB. Earth
and Planetary Science Letters, 179:9–20.
MAGNETIZATION, PIEZOREMANENCE AND
STRESS DEMAGNETIZATION
With the advent of paleomagnetic studies in the 1940s, scientists
began to wonder how the effect of stress, from burial, folding, etc.,
would influence the magnetic remanence of rocks. By the mid-
1950s, an intense research effort was underway aimed at understand-
ing piezoremanence, which is the remanent magnetization produced
by stress, as well as the opposite effect of stress demagnetization.
The laboratory experiments suggested that the magnetic signals of
rocks were sensitive to stresses typically found around fault zones;
and it was calculated that such stress-induced changes would in turn
modify the local magnetic field. Scientists thought that earthquakes
could be predicted by monitoring magnetic-field variations.
How pressure (stress) affects magnetic remanence
The origin of a spontaneous magnetic remanence is commonly asso-
ciated with electron exchange between iron (or other transition metals
such as Cr, Ni, etc.) atoms. For the iron oxide magnetite (Fe
3
O
4
), the
most abundant magnetic mineral in the Earth’s crust, the exchange is
indirect, passing through an oxygen atom. The spontaneous magnetiza-
tion of magnetite is generated in magnetic lattices established by the
crystallographic arrangement of the atoms. The sum of the magnetic
moments from the lattices comprises the net spontaneous magnetization
of the mineral. The bond lengths and bond angles between iron and oxy-
gen atoms of a particular lattice control the magnetic intensities and
directions of that lattice. Thus, when a stress acts on a material, the bond
lengths and angles change, which will in turn modify the lattices’ mag-
netic moments. This is why the nature of the stress, be it uniaxial, hydro-
static (equal on all sides), compressive, or tensile, can have important
consequences on the magnetic properties of materials. If a material com-
presses isotropically under a pure hydrostatic stress, bond lengths will
decrease, yet bond angles will remain unchanged; whereas, uniaxial or
shear stress will change the bond angles as well as the bond lengths.
Such changes depend on the volumetric compressibility of the magnetic
mineral. For example, the volume of magnetite decreases by about 2%
per gigapascal (GPa) (note that 1 GPa ¼ 10 kilobars (kbar); for the
Earth, 1 kbar corresponds to 3.5 km depth). This is why one needs very
large stresses to significantly modify the electronic configuration and
the magnetic lattice networks.
Size and shape are other factors influencing the magnetic properties
of materials. In extremely small grains, electron exchange exists, but
the spontaneous moment does not remain in a fixed direction within
the crystal due to thermal fluctuations, even at room temperature.
These grains are called superparamagnetic because they have no spon-
taneous moment in the absence of an external magnetic field, yet in the
presence of even a small external field, the randomizing thermal
energy is overcome, and the grains display magnet-like behavior. At
a critical size, about 0.05 mm for needle-shaped magnetite grains, the
spontaneous magnetic moment becomes fixed within the crystal, thus
resembling a dipolar magnet with a positive and a negative pole. Such
grains are called single domain. At even greater volumes, the magnetic
energy of the grain becomes too great and new magnetic domains grow
such that the magnetic vectors of the neighboring domains are oriented
in directions that diminish the overall magnetization of the crystal. These
are called multidomain grains. Multidomain grains often have a net
moment because the sum of the magnetic vectors of all the domains does
not exactly equal zero.
The magnetizations of single- and multidomain grains react differ-
ently to an imposed stress, just as they do with temperature or applied
fields. Hydrostatic stress may cause the net spontaneous moment in a
single-domain grain to reorient to a new position depending on the
grain’s shape, presence of crystal defects, etc. Under subhydrostatic
stress, the magnetization in a single-domain grain will rotate and
change in intensity because different crystallographic axes will experi-
ence different stresses, which will deform the exchange network.
Under any stress condition, the individual domains in a multidomain
grain may grow or shrink to compensate for the deformation, which
will modify the magnetic intensity and direction of the grain. Uniaxial
stresses are more efficient than hydrostatic stresses at causing domain
reorganization.
In sum, two processes contribute to the piezoremanent behavior of
materials, both of which can act independently or simultaneously at
a given stress condition. The first is a microscopic effect that modifies
the electron exchange couple, and the second is more of a macroscopic
effect where the magnetization becomes reoriented within a grain.
Each type of magnetic mineral and the domain states of each mineral
have their own particular piezoremanent and stress demagnetization
MAGNETIZATION, PIEZOREMANENCE AND STRESS DEMAGNETIZATION 599