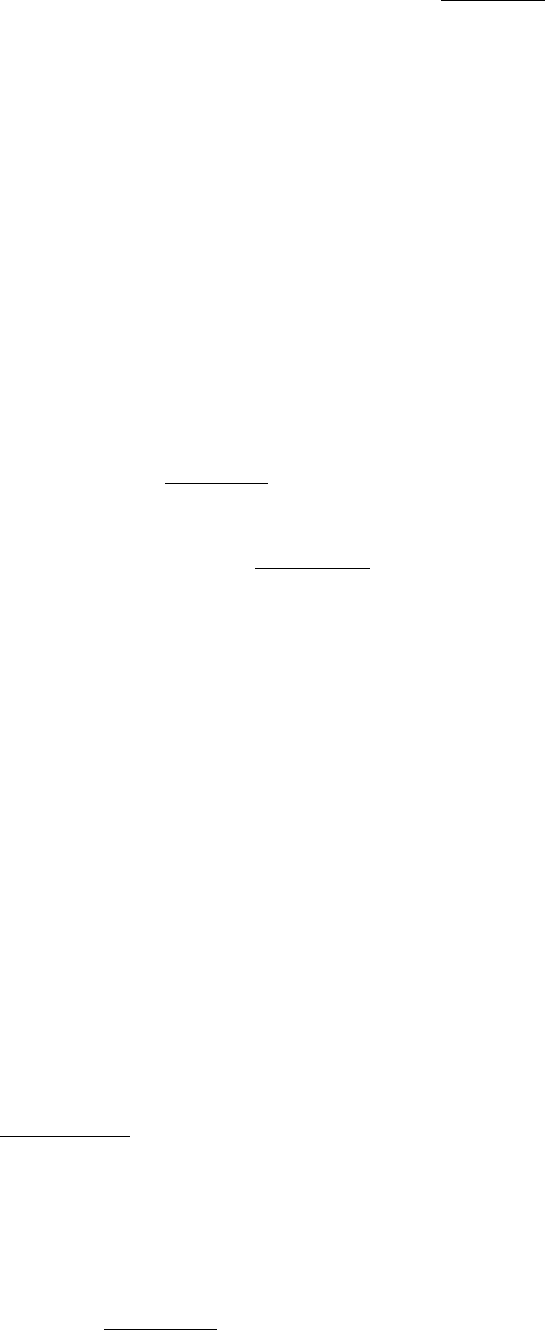
transmembrane helix. The structure was determined for a complex formed between G
α
s
bound to a GTP analog and
protein fragments corresponding to the active adenylate cyclase (Figure 15.8). As expected, the G protein binds to
adenylate cyclase through the surface that had bound the β γ dimer when the G protein was in its GDP form. The
activation of the G protein exposes this surface and subtly changes it so that it now binds the surface of adenylate cyclase
in preference to G
β
γ
. The interaction of G
α
s
with adenylate cyclase favors a more catalytically active conformation of
the enzyme, thus stimulating cAMP production. The net result is that the binding of epinephrine to the receptor on the
cell surface increases the rate of cAMP production inside the cell.
15.1.4. G Proteins Spontaneously Reset Themselves Through GTP Hydrolysis
The ability to shut down signal-transduction pathways is as critical as the ability to turn them on. How is the signal
initiated by activated 7TM receptors switched off? G
α
subunits have intrinsic GTPase activity, hydrolyzing bound GTP
to GDP and P
i
. This hydrolysis reaction is slow, however, requiring from seconds to minutes and thus allowing the GTP
form of G
α
to activate downstream components of the signal-transduction pathway before GTP hydrolysis deactivates
the subunit. In essence, the bound GTP acts as a built-in clock that spontaneously resets the G
α
subunit after a short
time period. After GTP hydrolysis and the release of P
i
, the GDP-bound form of G
α
then reassociates with G
β
γ
to
reform the heterotrimeric protein (Figure 15.9).
The hormone-bound activated receptor must be reset as well to prevent the continuous activation of G proteins. This
resetting is accomplished by two processes (Figure 15.10). First, the hormone dissociates, returning the receptor to its
initial, unactivated state. The likelihood that the receptor remains in its unbound state depends on the concentration of
hormone. Second, the hormone-receptor complex is deactivated by the phosphorylation of serine and threonine residues
in the carboxyl-terminal tail. In the example under consideration, β-adrenergic receptor kinase phosphorylates the
carboxyl-terminal tail of the hormone-receptor complex but not the unoccupied receptor. Finally, the binding of β -
arrestin, binds to the phosphorylated receptor and further diminishes its G-protein-activating ability. Phosphorylation
and the binding of β-arrestin account for the desensitization (adaptation) of the receptor subsequent to prolonged
exposure to epinephrine. The epinephrine-initiated cascade, like many other signal-transduction processes, has evolved
to respond to changes in the strength of stimuli rather than to their absolute level. Adaptation is advantageous because it
enables receptors to respond to changes in the level of stimuli over a wide range of background levels.
15.1.5. Cyclic AMP Stimulates the Phosphorylation of Many Target Proteins by
Activating Protein Kinase A
Let us continue to follow the information flow down this signal-transduction pathway. The increased concentration of
cAMP can affect a wide range of cellular processes. For example, it enhances the degradation of storage fuels, increases
the secretion of acid by the gastric mucosa, leads to the dispersion of melanin pigment granules, diminishes the
aggregation of blood platelets, and induces the opening of chloride channels. How does cAMP influence so many
cellular processes? Is there a common denominator for its diverse effects? Indeed there is. Most effects of cyclic AMP in
eukaryotic cells are mediated by activation of a single protein kinase. This key enzyme is called protein kinase A (PKA).
As discussed in Section 10.4.2, PKA consists of two regulatory (R) chains and two catalytic (C) chains. In the absence of
cAMP, the R
2
C
2
complex is catalytically inactive. The binding of cAMP to the regulatory chains releases the catalytic
chains, which are enzymatically active on their own. Activated PKA then phosphorylates specific serine and threonine
residues in many targets to alter their activity. The significance and far reach of the adenylate cyclase cascade are seen in
the following examples:
1. In glycogen metabolism (Section 21.5), PKA phosphorylates two enzymes that lead to the breakdown of this
polymeric store of glucose and the inhibition of further glycogen synthesis.
2. PKA stimulates the expression of specific genes by phosphorylating a transcriptional activator called the cAMP-