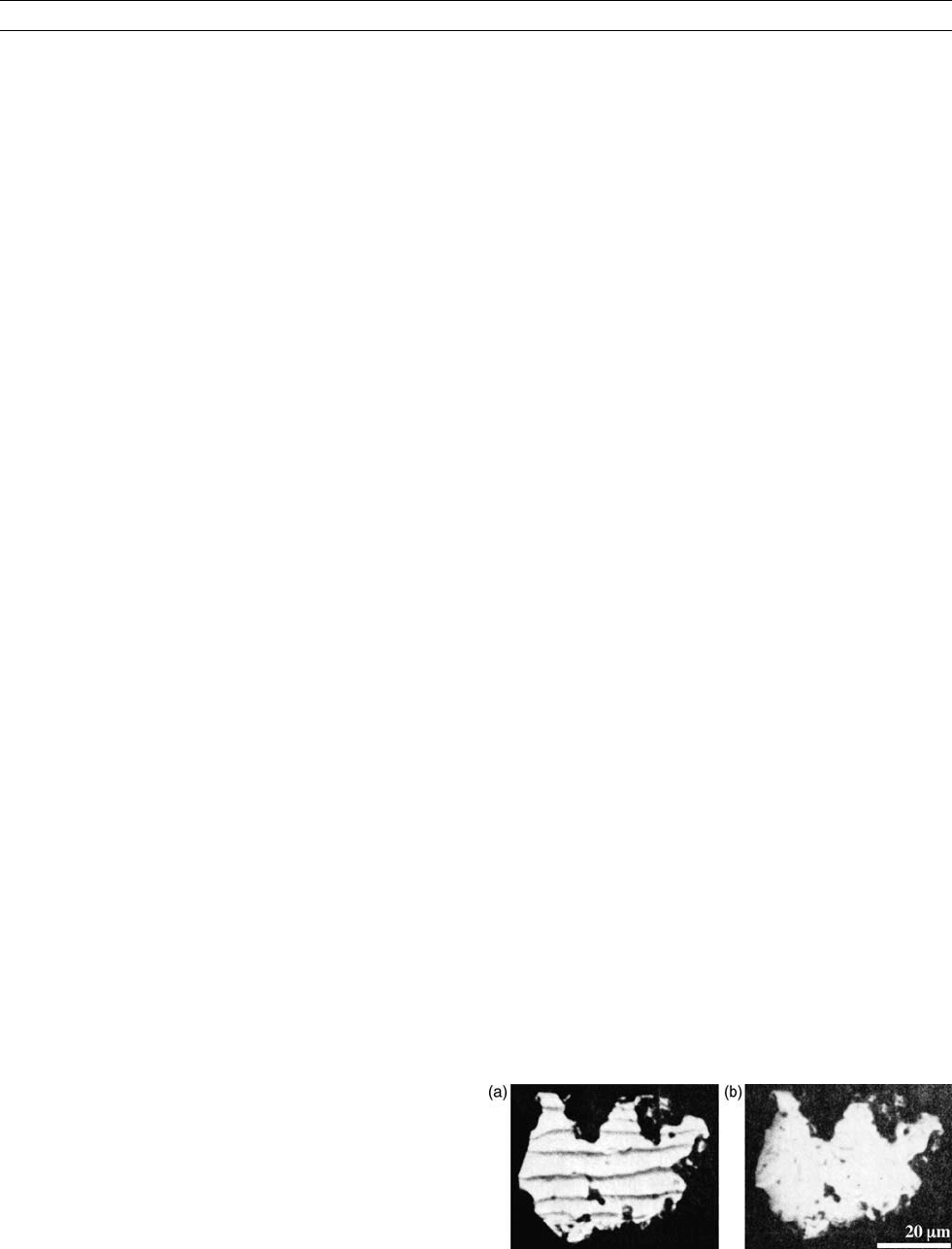
classical domain structures expected for magnetite; some models predict
“body” domains separated by domain walls, with closure domains at the
surface (e.g., Xu et al., 1994; Williams and Wright, 1998).
According to several micromagnetic models of hysteresis in submi-
cron magnetite, magnetization reversal can occur through LEM-LEM
transitions (e.g., flower to vortex state). Reversal can take place
through almost independent reversals of the particle’s core and outer
shell (e.g., Williams and Dunlop, 1995).
Dunlop et al. (1994) used 1D micromagnetic models to investi-
gate transdomain TRM. Transdomain TRM—that is, acquisition of
TRM through LEM-LEM transitions —had been proposed earlier by
Halgedahl (1991), who observed denucleation of walls and domains
in titanomagnetite during cooling (see below for a discussion of these
results). In particular, Halgedahl’s observations strongly suggested that
denucleation could give rise to SD-like TRMs in grains that, after
other magnetic treatments, contained domain walls. Furthermore,
Halgedahl (1991) found that a grain could “arrive” in a range of
LEM states after replicate TRM acquisitions.
To determine whether transdomain TRM could be acquired by
stress-free, submicron magnetite particles free of defects, Dunlop
et al. (1994) calculated the energy barriers for all combinations among
single domain-two-domain-three-domain transitions with decreasing
temperatures from the Curie point of magnetite in a weak external
field. They assumed that LEM-LEM transitions were driven by ther-
mal fluctuations across LEM-LEM energy barriers and that thermal-
equilibrium populations of LEM states were governed by Boltzmann
statistics. According to their results, after acquiring TRM most popula-
tions would be overwhelmingly biased toward GEM domain states,
and an individual particle should not exhibit a range of LEM states
after several identical TRM runs.
Using renormalization group theory, Ye and Merrill (1995) arrived
at a very different conclusion. According to their calculations, short-
range ordering of spins just below the Curie point could give rise to
a variety of LEM states in the same particle after replicate coolings.
These conflicting theoretical results are discussed below in the
context of experiments.
Domain observations
Methods of imaging domains and domain walls
Rock magnetists have mainly used three methods to image domains
and domain walls: the Bitter pattern method, the magneto-optical Kerr
effect (MOKE), and magnetic force microscopy (MFM). Two other
methods—transmission electron microscopy (TEM) and off-axis elec-
tron holography—have been used in a very limited number of studies
on magnetite.
The Bitter method images domain walls through application of a
magnetic colloid to a smooth, polished surface carefully prepared to
eliminate residual strain from grinding (e.g., see details in Halgedahl
and Fuller, 1983). Colloid particles are attracted by the magnetic field
gradients around walls, giving rise to Bitter patterns. When viewed
under reflected light, patterns appear as dark lines against a grain’s
bright, polished surface. With liquid colloid, this method can re-
solve details as small as about 1 mm. If colloid is dried on the sample
surface and the resultant pattern is viewed in a scanning electron
microscope (SEM), features as small as a few tenths of one micrometer
can be resolved (Moskowitz et al., 1988; Soffel et al., 1990).
The MOKE is based on the rotation of the polarization plane of inci-
dent light by the magnetization at a grain’s surface. Unlike the Bitter
method, the MOKE images domains themselves, rather than domain
walls. Domains appear as areas of dark and light, the result of their
contrasting magnetic polarities (Hoffmann et al., 1987; Worm et al.,
1991; Heider and Hoffmann, 1992; Ambatiello et al., 1999). The
MOKE has the same resolving power as the Bitter method.
In the MFM, a magnetized, needle-shaped tip is vibrated above the
highly polished surface of a magnetic sample. Voltage is induced in the
tip by the magnetic force gradients resulting from domains and domain
walls. The effects of any surface topography are removed by scanning
the surface with the nonmagnetic tip of an atomic force microscope.
The MFM can resolve magnetic features as small as 0.01 mm
(Williams et al., 1992; Proksch et al., 1994; Moloni et al., 1996;
Pokhil and Moskowitz, 1996, 1997; Frandson et al., 2004).
Styles of domains observe d in magnetic minerals of
paleomagnetic significance
In rock magnetism, the majority of domain observation studies have
focused on four magnetic minerals, all important to paleomagnetism:
pyrrhotite (Fe
7
S
8
), titanomagnetite of roughly intermediate composi-
tion (near Fe
2.4
Ti
6
O
4
, or TM60), magnetite (Fe
3
O
4
), and hematite
(Fe
2
O
3
).
Owing to its high magnetocrystalline anisotropy constant and rela-
tively weak magnetostriction constant, pyrrhotite behaves magnetically
as a uniaxial material. When studied with the Bitter method, pyr-
rhotite often exhibits fairly simple domain patterns, which suggest
lamellar domains separated by 180
walls (Figure M19a) (Soffel,
1977; Halgedahl and Fuller, 1983).
Despite being cubic, intermediate titanomagnetites rarely, if ever,
exhibit the arrays of closure domains, 71
, and 109
walls that
one would predict. Instead, these minerals usually display very com-
plex patterns of densely spaced, curved walls. Possibly, these complex
structures result from varying amounts of strain within the particle
(Appel and Soffel, 1984, 1985). Occasionally, grains exhibit simple
arrays of parallel walls, such as that shown in Figure M20 (e.g.,
Halgedahl and Fuller, 1980, 1981), but it is not unusual to observe
wavy walls alternating with rows of reverse spikes (Halgedahl, 1987;
Moskowitz et al., 1988). Both simple and wavy patterns suggest a
dominant, internal stress that yields a uniaxial anisotropy, although
the origin of this stress is still unclear. On one hand, it could originate
from the mechanical polishing required to prepare samples for domain
studies. Alternatively, in igneous rock samples stress could be gener-
ated during cooling, due to differences in the coefficients of thermal
expansion among the various minerals.
Small magnetite grains randomly dispersed in a rock or a synthetic
rock-like matrix generally display simple arrays of straight domain
walls, if the domains’ magnetizations are sufficiently close to being
parallel to the surface of view (e.g., Worm et al., 1991; Geiß et al.,
1996) (Figure M21 ). In such samples, however, there appears to be a
paucity of closure domains, perhaps the result of observation surfaces
being other than {100} planes.
In an early study by Bogdanov and Vlasov (1965), Bitter patterns of
71
and 109
walls were observed on cleavage planes of a few small
magnetite particles obtained by crushing a natural crystal. Similarly,
from Bitter patterns Boyd et al. (1984) found both closure domains
and networks of 180
,71
, and 109
walls in several magnetite parti-
cles in a granodiorite. Body domains accompanied by closure domains
were also reported by Smith (1980), who applied the TEM method to a
Figure M19 Bitter patterns on a particle of natural pyrrhotite
(a) after demagnetization in an alternating field of 1000 Oe and (b)
in an apparently SD-like state after acquiring saturation
remanence in 15 kOe.
MAGNETIC DOMAINS 495