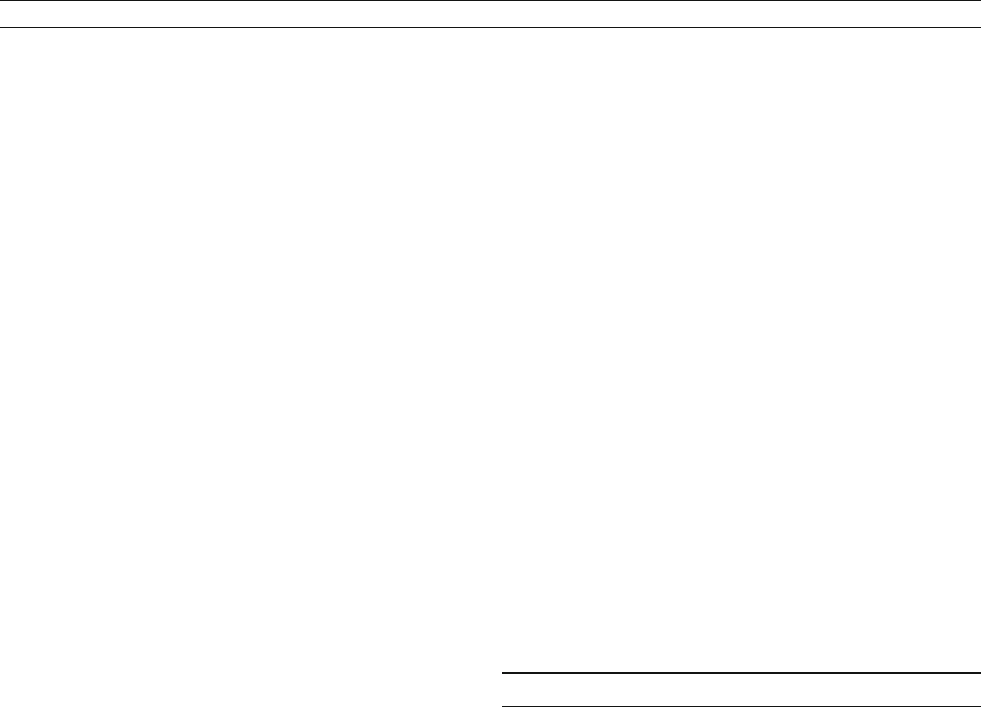
d
36
S
TFL
¼ 1:9 d
34
S ð4Þ
with data falling on a respective Terrestrial Fractionation Line
(TFL) in a three-isotope-plot. Multiple sulfur isotope analyses
of sedimentary sulfide and sulfate of Archean and early Paleo-
proterozoic age (>2350 million years) yielded clear deviations
from these mass-dependent relationships (e.g., Farquhar et al.,
2000). Such mass-independent fractionation (MIF) in a mea-
sured sample can be quantified as:
D
33
S ¼ d
33
S
measured
d
33
S
TFL
ð5Þ
D
36
S ¼ d
36
S
measured
d
36
S
TFL
ð6Þ
Based on experimental and modelling data, this mass-indepen-
dent isotope fractionation is believed to result from photoche-
mical reactions in the atmosphere involving gaseous sulfur
species, in particular sulfur dioxide. Furthermore, respective
reactions require the absence of an effective UV shield (like
ozone). Consequently, the presence of MIF-S has been taken
as evidence for the absence of free oxygen in the Archean
and early Paleoproterozoic atmosphere (e.g., Farquhar et al.,
2000; 2007). Modelling evidence suggests that the maximum
concentration of atmospheric oxygen had to be <10
5
of the
present atmospheric level (PAL) in order to yield MIF-S. Sedi-
mentary sulfur younger than 2350 million years displays only
mass-dependent sulfur isotopic fractionation, indicating that
the atmospheric oxygen level had increased to >10
2
PAL
(e.g., Pavlov and Kasting, 2002).
Harald Strauss and Ján Veizer
Bibliography
Canfield, D.E., 2001. Biogeochemistry of sulfur isotopes. In Valley, J.W.,
and Cole, D.R. (eds.), Stable Isotope Geochemistry. Rev. Mineral. Geo-
chem., 43, 607–636.
Canfield, D.E., and Raiswell, R., 1999. The evolution of the sulfur cycle.
Am. J. Sci., 299, 697–723.
Canfield, D.E., and Thamdrup, B., 1994. The production of
34
S-depleted
sulphide during bacterial disproportionation of elemental sulphur.
Science, 266, 1973–1975.
Canfield, D.E., Habicht, K.S., and Thamdrup, B., 2000. The Archean sulfur
cycle and the early history of atmospheric oxygen. Science, 288,
658–661.
Clark, I., and Fritz, P., 1997. Environmental Isotopes in Hydrogeology.
Boca Raton, FL: CRC Press, 328pp.
Claypool, G.E., Holser, W.T., Kaplan, I.R., Sakai, H., and Zak, I., 1980.
The age curve of sulfur and oxygen isotopes in marine sulfate and their
mutual interpretation. Chem. Geol., 28, 190–260.
Detmers, J., Brüchert, V., Habicht, K. S., and Kuever, J., 2001. Diversity of
sulphur isotope fractionations by sulphate-reducing prokaryotes. Appl.
Environ. Microbiol., 67, 888–894.
Farquhar, J., Bao, H. M., and Thiemens, M., 2000. Atmospheric influence
of Earth's earliest sulfur cycle. Science, 289, 756–758.
Farquhar, J., Peters, M., Johnston, D.T., Strauss, H., Masterson, A.,
Wiechert, U., and Kaufman, A.J., 2007. Isotopic evidence for
Mesoarchaean anoxia and changing atmospheric sulphur chemistry.
Nature, 449, 706–709.
Grinenko, V., and Krouse, H.R., 1992. Isotope data on the nature of river-
ine sulfates. Mitt. Geol.-Paläont. Inst. Univ. Hamburg, 72,9–18.
Habicht, K.S., and Canfield, D.E., 2001. Isotope fractionation by sulphate-
reducting natural populations and the isotopic composition of sulphides
in marine sediments. Geology, 29, 555–558.
Habicht, K.S., Canfield, D.E., and Rethmeier, J., 1998. Sulphur isotope
fractionation during bacterial sulphate reduction and disproportionation
of thiosulphate and sulfite. Geochim. Cosmochim. Acta, 62,
2585–2595.
Hoefs, J., 1997. Stable Isotope Geochemistry. Berlin: Springer, 201pp.
Holland, H.D., 2002. Volcanic gases, black smokers, and the great oxida-
tion event. Geochim. Cosmochim. Acta, 66, 3811–3826.
Holser, W.T., Schidlowski, M., Mackenzie, F.T., and Maynard, J.B., 1988.
Geochemical cycles of carbon and sulfur. In Gregor, C.B., Garrels, R.M.,
Mackenzie, F.T., and Maynard, J.B. (eds.), Chemical Cycles in the Evolu-
tion of the Earth. New York: Wiley, pp. 105–173.
Kampschulte, A., and Strauss, H., 2003. The sulphur isotopic evolution of
Phanerozoic seawater based on the analysis of structurally substituted
sulphate in carbonates. Chem. Geol.
, 204(3–4), 255–286.
Nielsen, H., 1989. Local and global aspects of the sulphur isotope age
curve of oceanic sulphate. In Brimblecombe, P., and Lein, A.Y. (eds.),
Evolution of the Global Biogeochemical Sulphur Cycle. New York:
Wiley, pp. 57–64.
Ohmoto, H., 1997. When did the Earth’s atmosphere become oxic? The
Geochemical News, 93-Fall,12–28.
Pavlov, A.A., Kasting, J.F., 2002. Mass-independent fractionation of sulfur
isotopes in Archean sediments: Strong evidence for an anoxic Archean
atmosphere. Astrobiology, 2,27–41.
Rees, C.E., Jenkins, W.J., and Monster, J., 1978. The sulphur isotopic com-
position of ocean water sulphate. Geochim. Cosmochim. Acta, 42,
377–381.
Strauss, H., 1999. Geological evolution from isotope proxy signals –
sulphur. Chem. Geol., 161,89–101.
Strauss, H., 2002. The isotopic composition of Precambrian sulphides –
seawater chemistry and biological evolution. Spec. Publ. Int. Assoc.
Sediment., 33,67–105.
Cross-references
Atmospheric Evolution, Earth
Carbon Isotope Variations over Geologic Time
Isotope Fractionation
Stable Isotope Analysis
SUN-CLIMATE CONNECTIONS
Introduction
All energy distributed in the climate system originates from
the Sun. Earth’s surface temperature is maintained by a balance
between incoming and outgoing radiation. Irradiance is the most
important parameter related to solar variability, as the relationship
between irradiance and climate in radiative balance is simple and
direct. The Earth currently receives an average of 1,367 W m
2
,
integrated over all wavelengths. Climate variability directly
associated with the radiative imbalance caused by changes in
solar radiation is “solar forced.” In contrast, a “solar influ-
enced” or “solar triggered” climate change depends mostly on
atmospheric or oceanic feedback mechanisms for the effect.
The Sun also generates strong magnetic fields. Through
their interaction with Earth’s magnetic field and charged parti-
cles such as cosmic rays, magnetic fields are central to both
observational and proxy records establishing solar variability.
A current challenge in Sun-climate research is the interpreta-
tion of long-term proxy records related to the Sun’s magnetic
activity. It will only be possible to confirm a Sun-climate
connection for past climates when the innermost workings of
the Sun are understood well enough to quantify the relation
between magnetic proxies and irradiance.
On millennial and shorter time scales, there is evidence
that small changes in irradiance affect climate. It has proven
difficult to identify the physical mechanisms by which small
observed and reconstructed irradiance changes (a few tenths
of a percent) could produce observed and reconstructed climate
changes, up to 2
C within 50 years (Bond et al., 2001). Over
SUN-CLIMATE CONNECTIONS 929