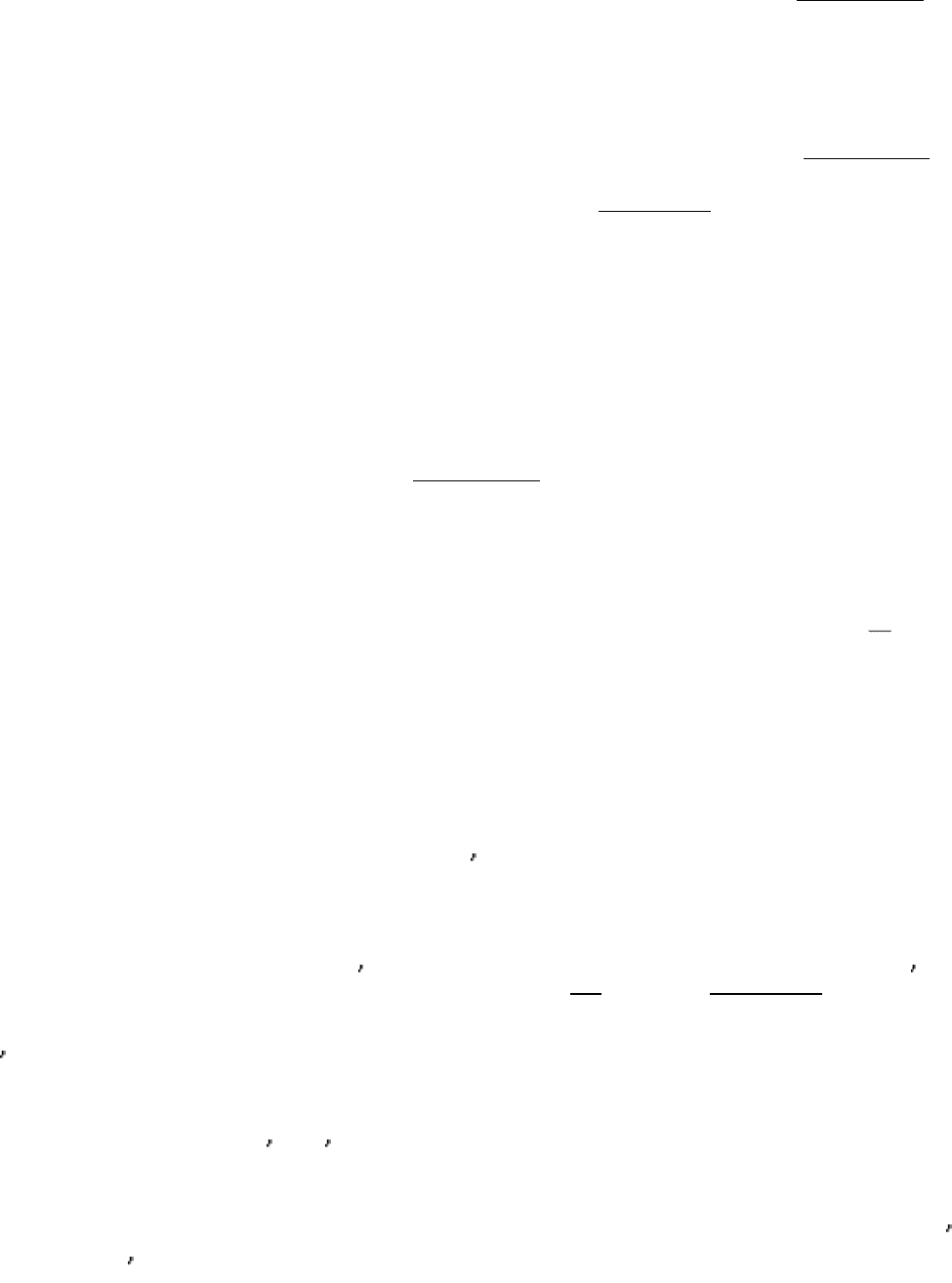
already discussed. Apolipoprotein B (apo B) plays an important role in the transport of triacylglycerols and cholesterol
by forming an amphipathic spherical shell around the lipids carried in lipoprotein particles (Section 26.3.1). Apo B exists
in two forms, a 512-kd apo B-100 and a 240-kd apo B-48. The larger form, synthesized by the liver, participates in the
transport of lipids synthesized in the cell. The smaller form, synthesized by the small intestine, carries dietary fat in the
form of chylomicrons. Apo B-48 contains the 2152 N-terminal residues of the 4536-residue apo B-100. This truncated
molecule can form lipoprotein particles but cannot bind to the low-density-lipoprotein receptor on cell surfaces. What is
the biosynthetic relation of these two forms of apo B? One possibility a priori is that apo B-48 is produced by proteolytic
cleavage of apo B-100, and another is that the two forms arise from alternative splicing (see Section 28.3.6). The results
of experiments show that neither occurs. A totally unexpected and new mechanism for generating diversity is at work:
the changing of the nucleotide sequence of mRNA after its synthesis (Figure 28.26). A specific cytidine residue of mRNA
is deaminated to uridine, which changes the codon at residue 2153 from CAA (Gln) to UAA (stop). The deaminase that
catalyzes this reaction is present in the small intestine, but not in the liver, and is expressed only at certain developmental
stages.
RNA editing is not confined to apolipoprotein B. Glutamate opens cation-specific channels in the vertebrate central
nervous system by binding to receptors in postsynaptic membranes. RNA editing changes a single glutamine codon
(CAG) in the mRNA for the glutamate receptor to the codon for arginine (read as CGG). The substitution of Arg for Gln
in the receptor prevents Ca
2+
, but not Na
+
, from flowing through the channel. RNA editing is likely much more common
than was previously thought. The chemical reactivity of nucleotide bases, including the susceptibility to deamination that
necessitates complex DNA-repair mechanisms (Section 27.6.3), has been harnessed as an engine for generating
molecular diversity at the RNA and, hence, protein levels.
In trypanosomes (parasitic protozoans), a different kind of RNA editing markedly changes several mitochondrial
mRNAs. Nearly half the uridine residues in these mRNAs are inserted by RNA editing. A guide RNA molecule identifies
the sequences to be modified, and a poly(U) tail on the guide donates uridine residues to the mRNAs undergoing editing.
It is evident that DNA sequences do not always faithfully disclose the sequence of encoded proteins
functionally
crucial changes to mRNA can take place.
28.3.3. Splice Sites in mRNA Precursors Are Specified by Sequences at the Ends of
Introns
Most genes in higher eukaryotes are composed of exons and introns. The introns must be excised and the exons linked to
form the final mRNA in a process called splicing. This splicing must be exquisitely sensitive: a one-nucleotide slippage
in a splice point would shift the reading frame on the 3
side of the splice to give an entirely different amino acid
sequence. Thus, the correct splice site must be clearly marked. Does a particular sequence denote the splice site? The
base sequences of thousands of intron- exon junctions within RNA transcripts are known. In eukaryotes from yeast to
mammals, these sequences have a common structural motif: the base sequence of an intron begins with GU and ends
with AG. The consensus sequence at the 5
splice in vertebrates is AGGUAAGU (Figure 28.27). At the 3 end of an
intron, the consensus sequence is a stretch of 10 pyrimidines (U or C), followed by any base and then by C, and ending
with the invariant AG. Introns also have an important internal site located between 20 and 50 nucleotides upstream of the
3
splice site; it is called the branch site for reasons that will be evident shortly. In yeast, the branch site sequence is
nearly always UACUAAC, whereas in mammals a variety of sequences are found.
Parts of introns other than the 5
and 3 splice sites and the branch site appear to be less important in determining where
splicing takes place. The length of introns ranges from 50 to 10,000 nucleotides. Much of an intron can be deleted
without altering the site or efficiency of splicing. Likewise, splicing is unaffected by the insertion of long stretches of
DNA into the introns of genes. Moreover, chimeric introns crafted by recombinant DNA methods from the 5
end of one
intron and the 3
end of a very different intron are properly spliced, provided that the splice sites and branch site are
unaltered. In contrast, mutations in each of these three critical regions lead to aberrant splicing.
Despite our knowledge of splice-site sequences, predicting splicing patterns from genomic DNA sequence information