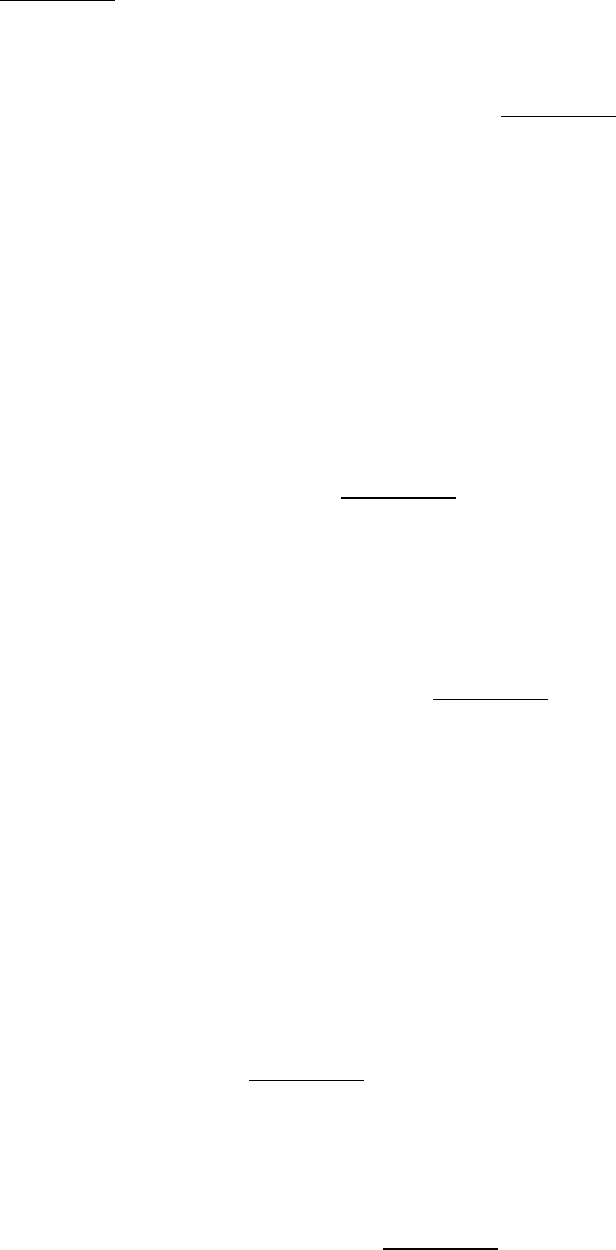
L, or loose, conformation. This conformation binds ADP and P
i
. It, too, is sufficiently constrained that it cannot release
bound nucleotides. The final subunit will be in the O, or open, form. This form can exist with a bound nucleotide in a
structure that is similar to those of the T and L forms, but it can also convert to form a more open conformation and
release a bound nucleotide (Figure 18.31). This structure, with one of the three β subunits in an open, nucleotide-free
state, as well as one with one of the β subunits in a nucleotide-bound O conformation, have been observed
crystallographically.
The interconversion of these three forms can be driven by rotation of the γ subunit (Figure 18.32). Suppose the γ subunit
is rotated 120 degrees in a counterclockwise direction (as viewed from the top). This rotation will change the subunit in
the T conformation into the O conformation, allowing the subunit to release the ATP that has been formed within it. The
subunit in the L conformation will be converted into the T conformation, allowing the transition of bound ADP + P
i
into
ATP. Finally, the subunit in the O conformation will be converted into the L conformation, trapping the bound ADP and
P
i
so that they cannot escape. The binding of ADP and P
i
to the subunit now in the O conformation completes the cycle.
This mechanism suggests that ATP can be synthesized by driving the rotation of the γ subunit in the appropriate
direction. Likewise, this mechanism suggests that the hydrolysis of ATP by the enzyme should drive the rotation of the γ
subunit in the opposite direction.
18.4.3. The World's Smallest Molecular Motor: Rotational Catalysis
Is it possible to observe the proposed rotation directly? Elegant experiments were performed with the use of a simple
experimental system consisting of cloned α
3
β
3
γ subunits only (Figure 18.33). The β subunits were engineered to
contain amino-terminal polyhistidine tags, which have a high affinity for nickel ions. This property of the tags allowed
the α
3
β
3
assembly to be immobilized on a glass surface that had been coated with nickel ions. The γ subunit was linked
to a fluorescently labeled actin filament to provide a long segment that could be observed under a fluorescence
microscope. Remarkably, the addition of ATP caused the actin filament to rotate unidirectionally in a counterclockwise
direction. The γ subunit was rotating, being driven by the hydrolysis of ATP. Thus, the catalytic activity of an individual
molecule could be observed. The counterclockwise rotation is consistent with the predicted mechanism for hydrolysis
because the molecule was viewed from below relative to the view shown in Figure 18.32.
More detailed analysis in the presence of lower concentrations of ATP revealed that the γ subunit rotates in 120-degree
increments, with each step corresponding to the hydrolysis of a single ATP molecule. In addition, from the results
obtained by varying the length of the actin filament and mea-suring the rate of rotation, the enzyme appears to operate
near 100% efficiency; that is, essentially all of the energy released by ATP hydrolysis is converted into rotational
motion.
18.4.4. Proton Flow Around the c Ring Powers ATP Synthesis
The direct observation of rotary motion of the γ subunit is strong evidence for the rotational mechanism for ATP
synthesis. The last remaining question is: How does proton flow through F
0
drive the rotation of the γ subunit? Howard
Berg and George Oster proposed an elegant mechanism that provides a clear answer to this question. The mechanism
depends on the structures of the a and c subunits of F
0
(Figure 18.34). The structure of the c subunit was determined both
by NMR methods and by x-ray crystallography. Each polypeptide chain forms a pair of α helices that span the
membrane. An aspartic acid residue (Asp 61) is found in the middle of the second helix. When Asp 61 is in contact with
the hydrophobic part of the membrane, the residue must be in the neutral aspartic acid form, rather than in the charged,
aspartate form. From 9 to 12 c subunits assemble into a symmetric membrane-spanning ring. Although the structure of
the a subunit has not yet been experimentally determined, a variety of evidence is consistent with a structure that
includes two proton half-channels that do not span the membrane (see Figure 18.34). Thus, protons can pass into either
of these channels, but they cannot move completely across the membrane. The a subunit directly abuts the ring
comprising the c subunits, with each half-channel directly interacting with one c subunit.