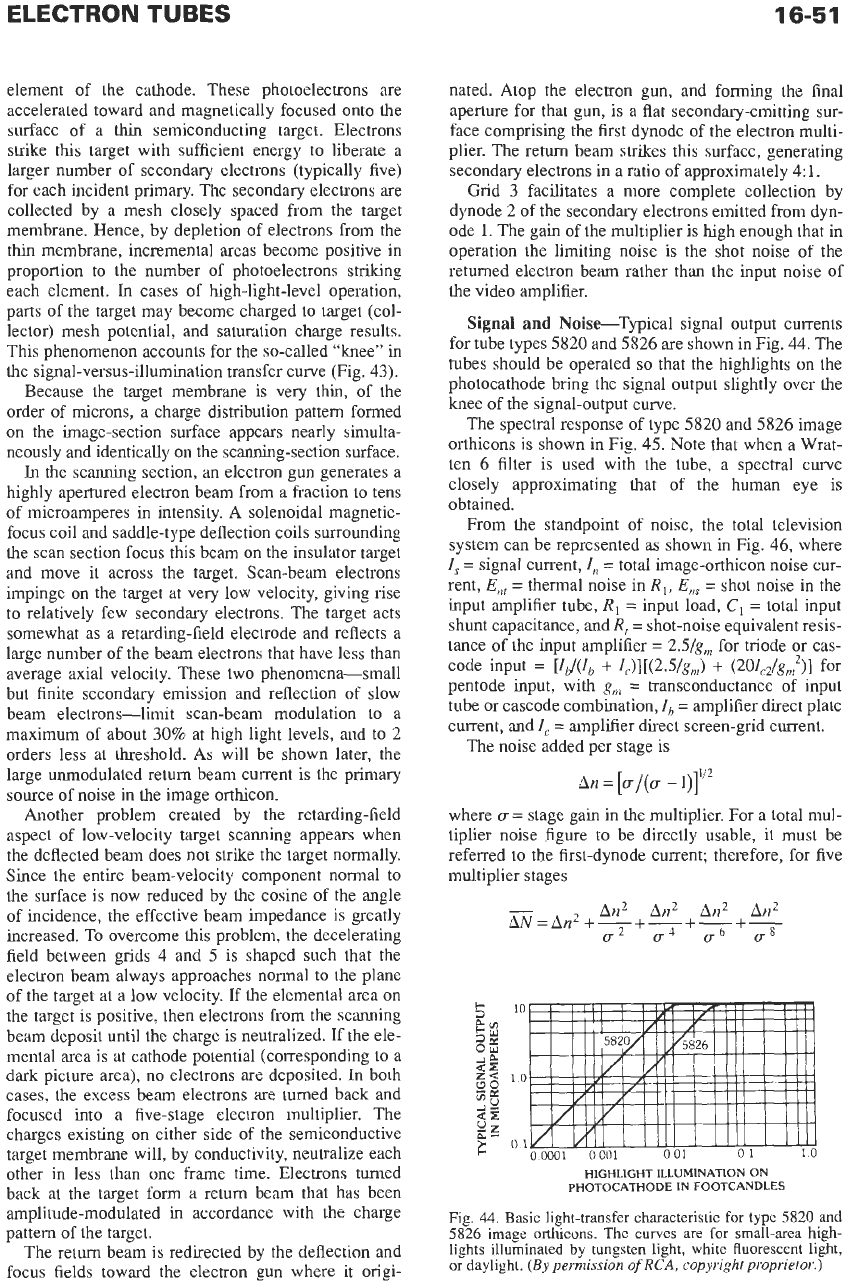
16-51
element of the cathode. These photoelectrons are
accelerated toward and magnetically focused onto the
surface of a thin semiconducting target. Electrons
strike this target with sufficient energy to liberate a
larger number of secondary electrons (typically five)
for each incident primary. The secondary electrons are
collected by a mesh closely spaced from the target
membrane. Hence, by depletion of electrons from the
thin membrane, incremental areas become positive in
proportion to the number of photoelectrons sh-iking
each element.
In
cases of high-light-level operation,
parts of the target may become charged to target (col-
lector) mesh potential, and saturation charge results.
This phenomenon accounts for the so-called “knee” in
the signal-versus-illumination transfer curve (Fig. 43).
Because the target membrane is very thin, of the
order of microns, a charge distribution pattern formed
on the image-section surface appears nearly simulta-
neously and identically
on
the
scanning-section surface.
In
the scanning section, an electron gun generates a
highly apertured electron beam from a fraction to tens
of microamperes in intensity.
A
solenoidal magnetic-
focus coil and saddle-type deflection coils surrounding
the scan section focus this beam on the insulator target
and move it across the target. Scan-beam electrons
impinge on the target at very low velocity, giving rise
to relatively few secondary electrons. The target acts
somewhat as a retarding-field electrode and reflects a
large number of the beam electrons that have less than
average axial velocity. These two phenomena-small
but finite secondary emission and reflection
of
slow
beam electrons-limit scan-beam modulation
to
a
maximum of about 30% at high light levels, and to 2
orders less at threshold. As will be shown later, the
large unmodulated return beam current is the primary
source of noise in the image orthicon.
Another problem created by the retarding-field
aspect of low-velocity target scanning appears when
the deflected beam does not strike the target normally.
Since the entire beam-velocity component normal to
the surface is now reduced by
the
cosine of the angle
of incidence, the effective beam impedance is greatly
increased. To overcome this problem, the decelerating
field between grids 4 and
5
is shaped such that the
electron beam always approaches normal to the plane
of the target at a low velocity. If the elemental area on
the target is positive, then electrons from the scanning
beam deposit until the charge is neutralized. If the ele-
mental area is at cathode potential (corresponding
to
a
dark picture area), no electrons are deposited.
In
both
cases, the excess beam electrons are turned back and
focused into a five-stage electron multiplier. The
charges existing on either side of the semiconductive
target membrane will, by conductivity, neutralize each
other in less than one frame time. Electrons turned
back at the target form a return beam that has been
amplitude-modulated
in
accordance with the charge
pattern of the target.
The return beam is redirected by the deflection and
focus fields toward the electron gun where it origi-
nated. Atop the electron gun, and forming the final
aperture for that gun, is a flat secondary-emitting sur-
face comprising the first dynode of the electron multi-
plier. The return beam strikes this surface, generating
secondary electrons in a ratio of approximately 4:
1.
Grid 3 facilitates a more complete collection by
dynode
2
of the secondary electrons emitted from dyn-
ode
1.
The gain of the multiplier is high enough that in
operation the limiting noise is the shot noise of the
returned electron beam rather than the input noise of
the video amplifier.
Signal
and
Noise-Typical signal output currents
for tube types 5820 and 5826
are
shown in Fig. 44. The
tubes should be operated
so
that the highlights on the
photocathode bring the signal output slightly over
the
knee of the signal-output curve.
The spectral response of type 5820 and 5826 image
orthicons is shown in Fig. 45. Note that when a Wrat-
ten
6
filter is used with the tube, a spectral curve
closely approximating that of the human eye is
obtained.
From the standpoint of noise, the total television
system can be represented as shown in Fig.
46,
where
Z,
=
signal current,
Z,
=
total image-orthicon noise cur-
rent,
E,,
=
thermal noise in
R,, E,,
=
shot noise in the
input amplifier tube,
R,
=
input load,
C,
=
total input
shunt capacitance, and
R,
=
shot-noise equivalent resis-
tance of the input amplifier
=
2.5/g,,1 for triode or cas-
code input
=
[Ib/(Zb
+
Zc)][(2.5/gnl)
+
(20Ic2/g,2)] for
pentode input, with g,
=
transconductance of input
tube or cascode combination,
Zb
=
amplifier direct plate
current, and
I,
=
amplifier direct screen-grid current.
The noise added per stage is
An
=
[(T/((T
-
l)]”’
where
(T
=
stage gain in
the
multiplier. For a total mul-
tiplier noise figure to be directly usable, it must be
referred to the first-dynode current; therefore, for five
multiplier stages
-
An2 An2 An2 An2
Ah’
=
An2
+-+-+-
+-
u2
u4
u6 u8
5
10
2:
a::
$!2
gg
<?
sc
5:
0
1
z*
10
VIIWI
Ill1
I
/Ill
I
Ill
00001
0001
0
01
01
10
HIGHLIGHT ILLUMINATION ON
PHOTOCATHODE IN FOOTCANDLES
Fig.
44.
Basic light-transfer characteristic for
type
5820
and
5826
image orthicons. The curves
are
for
small-area high-
lights illuminated
by
tungsten light, white fluorescent light,
or daylight.
(By
permission
of
RCA,
copyright proprietor.)